[mashshare]
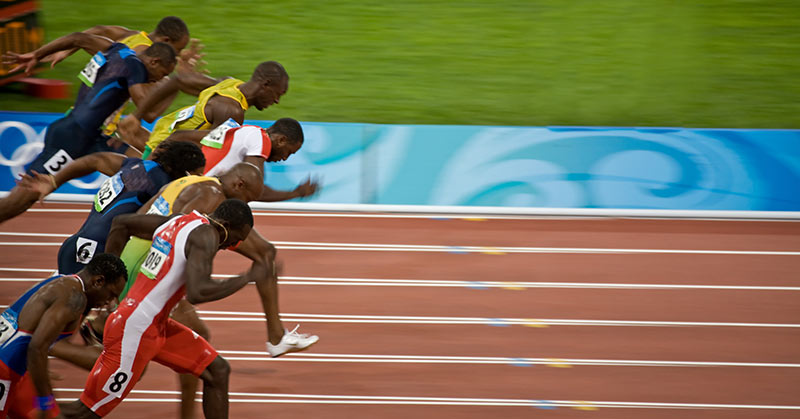
This article has been written from a “first principles” perspective, in order to objectively explain the dynamic and physiological structures of the preparation of a 100-meter sprinter. This is Part 1 of a two-part series. Part 2 in the series covers Sprint Weight Training Considerations and Temporal Placement.
Determining the Overall Performance Level of a Sprinter
The overall performance level of a sprinter is objectively quantified by their competition PB factored against the remainder of their known levels of biomotor output (power, strength, etc.), all along the continuum of specific to general motion. It is most important to understand the force-velocity profile of the sprinter, while they are sprinting.
No sensible discussion in this regard may begin, however, before the dynamics and physiology of the sprint motion are understood, as these are the reference points that provide meaning to any aspect of preparation. Alternatively, the attempt to prepare for an objective that is not clearly understood, despite the fact that there is a great amount already known about the objective, cannot take place in any sort of cogent discussion.
Biodynamic Considerations
A general overview of the dynamics of a sprint—the 100m event, for example—is as follows:
Block Start
The sprinter positions themself in the starting blocks so as to optimize their joint positions relative to their state of preparation and propulsive ability (biomotor output).
Universally important for all sprinters, however (regardless of their stage of preparation) is that:
- Their lead foot is positioned behind the plum line of the crease in the same side hip.
- Block clearance concludes with relative complete extension through the hips, knees, and ankles. If the front foot is positioned in front of the vertical axis of the crease in the hip, the distance the hips must travel in order to reach full extension is increased. Alternatively, if the front foot is positioned too far behind the hip, the distance the hips have to travel to reach extension is too short and thereby inhibits the propulsive force capability of the legs and backside muscles.
- Their hips are positioned above the horizontal axis of their shoulders. The extension of the back and hips plays a substantial role in the propulsive force required to achieve extension out of the blocks (as fast as possible). If the hips are positioned below the level of the shoulders, the angular displacement required for the hips to achieve extension is reduced, thereby minimizing the achievable exit velocity. Alternatively, as the hips are positioned higher and higher, relative to the shoulders (by way of increasing the horizontal distance between the hands), the neuromuscular demand placed upon the spinal erectors and gluteals becomes greater and greater.
- All things being equal, most sprinters, regardless of level, will benefit from positioning their shoulders exactly above, or marginally in front of, their hands, in the set position. Positioning the shoulders too far forward of the hands increases the amplitude of lead arm travel during take-off and the percentage of body mass that is supported by the hands in set position.
Acceleration
This is the phase of the sprint that begins with block clearance and ends when the sprinter reaches their maximum velocity (in which acceleration ceases to occur).
The fastest sprinters achieve faster 10m splits up to the point of max velocity and the total distance they accelerate is longer than their slower counterparts (they reach max velocity farther from the starting line).
- By any objective measure, the mechanical efficiency of a sprinter’s acceleration is characterized by the smoothest (most gradual/incremental) possible kinematic transition from the position in the blocks to the full upright sprint position. This is a point that has well been expounded upon by ALTIS Head Coach Shanghai Pierre-Jean Vazel.
- With each step during acceleration, the angle created by the sprinter’s extension relative to the track becomes incrementally greater.
- The physical sensation of accelerating is pronounced resultant of the muscle/mechanical nature of output required to overcome the sprinter’s static inertia and increase their rate of acceleration. The muscles are working very hard and the increased GCT (and subsequent longer time of applied force), in comparison to max velocity, renders a situation in which the actual “feeling” of acceleration is distinct from max velocity.
- The entire spine from, skull to sacrum, is well-served to remain in the anatomical position from the first step to the finish line. This is most obviously indicated by the sprinter’s head position.
- If the sprinter prematurely raises their head, they will extend their cervical spine. Alternatively, if the sprinter forces their chin/head down, they will flex their cervical spine. While there is no statistically significant data demonstrating that minor cervical rotations in either direction have mentionable implications on acceleration, the preservation of the anatomical position remains a viable model.
- In this way, the sprinter’s line of sight, if you imagine Superman-like lasers emitting from their eyes, should be relatively perpendicular to the long axis of their spine. They should not become parallel with the track until the sprinter is in the upright position; in which the long axis of their spine is relatively perpendicular to the track.
- The angle of extension itself, relative to the track, must be dependent upon each sprinter’s neuromuscular output ability.
- As the angle of extension, relative to the horizontal, becomes less and less, the sprinter must necessarily possess greater propulsive force output ability (in the associated muscles of the back, hips, and legs) in order to prevent falling on their face while attempting to accelerate.
- For this reason, forcing an unnaturally low position of acceleration is in no way a useful tactic for any sprinter whose biomotor preparation is insufficient to optimize a low angle of extension. In such attempts, the sprinter’s lack of sufficient propulsive output renders their effort to “stay low and drive” inefficient because they are bent forward at the waist at toe-off.
- Alternatively, a sprinter who rises to the upright position too soon may truncate the attainable acceleration due to the increased demand placed upon the legs to accelerate amidst too great an angle of extension, relative to the horizontal.
- Positive Shin Angles
- The relationship between the knee of the lead leg and the ground, when the foot makes contact, represents the degree of mechanical advantage that is achieved during early acceleration. An accepted rule is that the athlete maintains positive shin angles for the first four to five steps of an acceleration [10]. This kinematic position of the shank most effectively facilitates the delivery of force in the horizontal direction upon ground contact when the angle of the athlete’s extension is increasing from block clearance.
- Each foot strike should occur in relative approximation to the vertical axis created through the sprinter’s center of mass (just in front of bottom dead center).
- The farther in front of the hips the foot makes contact, the longer the duration the foot will be on the ground, as the hips must pass over the foot before the recovery cycle of that leg begins.
- The ankle/foot is the end-stage transmitter of force upon ground contact.
- The stiffness of the foot/ankle, and associated musculotendinous structures, is characterized by their resistance to deformation.
- The less motion that occurs about the ankle during GCT, the lesser the loss of energy as heat and the greater its kinetic transfer.
- The sprinter dorsi flexes the first metatarsal prior to GCT, which facilitates the approximate anatomical position of the ankle prior to GCT. This generates a pre-stretch on the Achilles/gastroc that contributes to greater elastic return during GCT and, subsequently, shorter ground contact times.
- During front-side action, the motion of the knees should be thought of as forward, and not upward.
- As the optimal ground strike is marginally in front of bottom dead center, the more the sprinter rotates their femur upwards, the greater the “artificial” increase in stride length by increasing the duration of the recovery cycle; not due to greater force application during GCT, but via the increase of the amplitude of femoral rotation beyond what is optimal.
- In effect, exaggerated knee lift, regardless of whether it occurs during acceleration or in the upright position, begins to shift the sprint action into a bound in which ground contact times are proportionally longer. The result is a reduction in horizontal velocity.
- During the recovery phase, the sprinter should collapse the knee such that the lower leg is relatively parallel to the horizontal axis when it is under the hips.
- When this is achieved, the view from behind the sprinter, at the moment in which the lower leg is parallel to the horizontal axis, will reveal the entire sole of the shoe—the bottom of the foot is relatively perpendicular to the horizontal axis.
- The position of the pelvis is of principle importance during all phases of the 100m event.
- o If the pelvis is anteriorly rotated, relative to its anatomical position, the sprinter necessarily experiences greater amplitude backside leg motion, as made evident by the foot travelling farther behind and upwards following toe-off. This is informally referred to as “kicking out the back.” This is the net result of the curvilinear “apparatus” of the sprinter’s leg cycling (envisage this as an orbital ellipse); being rotated in the direction they are sprinting.
- o When this occurs, ground contact occurs farther in front of the hips resultant of their negative vertical displacement due to their anteriorly rotated position. This results in a longer ground contact patch, as well as greater braking ground contact forces directed at the musculotendinous structures of the posterior knee and distal hamstring.
- The action of the arms is one of the most consequential motion dynamics in sprinting.
- The hips naturally rotate, due to the momentum generated by the legs moving back and forth. The arms provide counter rotation, about the thoracic spine to the rotation of the hips, and this contributes to the sprinter’s momentum of forward locomotion.
- The dynamics of the arms themselves deserves special attention:
- The length of the lever, represented by the sprinter’s arms, is defined by the degree to which the elbow is flexed and in reference to the hands moving back and forth in a curvilinear path of motion. (Similarly, if we consider the sprinter’s hands to represent the point of applied force, then the lever arm is described by the distance between the center of the rotating axis of the glenohumeral joint and the hands. Alternatively, if we consider the lever arm of any of the muscles that move the humerus, then we must measure the distance between the center of the rotating axis of the glenohumeral joint and the attachment points on the humerus of the respective muscle’s tendon attachment.)
- The greater the degree of elbow rotation (flexion), the shorter the lever and less mechanical energy required to move the arms back and forth. Alternatively, the lesser of a degree of elbow rotation (keeping the elbow extended) lengthens the lever, requiring more mechanical energy to move the arms back and forth.
- As the dynamics of faster and faster sprinting include higher ground contact forces delivered in less and less time, you may observe the interplay between force and velocity, as they are intrinsic to the sprint action. For this reason, while a fully extended elbow allows the arm to generate more force, and thereby momentum, during the back swing, for example, it requires more time to do so. Alternatively, a fully flexed elbow during the backswing rotates more quickly yet produces less force, and momentum. For these reasons, a near-uniform observation of the world’s fastest 100m sprinters reveals that the angle of the elbow closes just beyond 90 degrees during front-side action and opens slightly more beyond 90 degrees during back-side action.
- The sprinter must overcome their static inertia in the blocks. Thus, the time of force application associated with ground contact force becomes shorter and shorter, up until the moment the sprinter reaches maximum velocity. Correspondingly, the greatest degree of elbow extension during backside arm action, and overall motion about the shoulder, is observed during block clearance and the initial steps of acceleration.
Maximum Velocity
- The sprinter reaches the full upright position.
- Ground contact times are the shortest (~.08sec for the fastest males).
- Locomotive velocity is the highest (+12m/s for the fastest males).
- Muscle contractile velocity is the highest.
- The period of maximum velocity is generally limited to a distance of 10m, and close to 20m in some of the elites.
- The vast proportion of motion dynamics referenced during acceleration hold true in the upright position, with the most significant distinction being the angle of the sprinter’s extension at toe-off (relative to the horizontal).
- As the sprinter has achieved the full upright position and their maximum velocity, what began as a higher proportion of muscle/mechanical work during early acceleration has now transitioned to greater reactive/elastic contribution. The physical sensation of max velocity sprinting is much different than early acceleration, as the time of applied force into the track is less than one-tenth of a second.
- During the front side action of the legs, knee height is generally optimized when the horizontal distance between the knee and hip is maximized (~90 degrees of hip flexion).
- If the femur rotates upward, reducing the angle at the hip, the sprinter runs the risk of losing hip height as a result of a break in the knee of the stance leg during ground support.
- Alternatively, if the femur fails to rotate to a position parallel with the horizontal axis, the stride length will be shortened.
- Whereas, relative extension through the hip and knee is achieved at toe-off.
- Upon toe-off, the greater the athlete’s ability to collapse the knee of the recovery leg, the greater their ability to mitigate the mechanical cost of bringing the leg forward. In effect, the sprinter is creating a shorter lever. When this is achieved, the result is the lower leg becomes relatively parallel to the horizontal axis as the knee comes forward, and this transitions into the foot of the recovery leg passing at or above the level of the knee of the support leg. Hence the term “toe over knee” [10].
- Despite the fact that the sprinter is in the full upright position, the greatest distinguishing characteristics of their dynamics at this point, relative to lesser talented and/or developed sprinters, is the horizontal ground reaction force vector and impulse of their foot strike [5].
Speed Endurance
Speed endurance, or your preferred colloquial equivalent, describes the period following the conclusion of maximum velocity that characterizes the sprinter’s ability to sustain the highest percentage of their maximum velocity through to the finish.
- The preservation of mechanical optimization is of the utmost importance through this period in which the sprinter’s internal physiological environment becomes increasingly less conducive to generating top speed.
- Speed endurance will be expounded upon in the next section.
Physiological Considerations
The two primary bioenergetic domains (anaerobic and aerobic) are differentiated based upon the biochemical substrates that they metabolize in order to synthesize adenosine triphosphate (ATP), which is essential to facilitate muscle contraction. Simply put, the human organism has two primary ways of synthesizing ATP—with and without oxygen.
To be taken literally, the (an)aerobic system conducts its operations void of oxygen. This system is subdivided into the anaerobic-alactic (no lactic acid) and anaerobic-lactic (with lactic acid) —(or glycolytic reflective of the process of anaerobic glycolysis: the breakdown of glucose via the anaerobic machinery).
The anaerobic-alactic system is recognized as the short-term system, or the ATP-CP system in reference to the breakdown of creatine phosphate (CP), whose energy release couples with other processes specific to the re-synthesis of adenosine triphosphate (ATP). This system, regarding continuous movement, is responsible for the shortest-duration and highest-intensity muscular outputs.
The anaerobic-lactic system, the medium term system, signifies the process of anaerobic glycolysis. Glycolysis refers to the breakdown of glucose (sugar), and the subsequent energy release is one of the mechanisms associated with ATP synthesis. Lactic acid is one by-product in the process of anaerobic glycolysis; hence, the anaerobic-lactic system. In the context of continuous movement, this system is responsible for medium duration and relatively high intensity muscular output [8].
While at no point during human motion is any single bioenergetic resource solely responsible for movement, in a 100m sprint, the overwhelming bioenergetic contribution stems from anaerobic alactic and anaerobic lactic processes. It is a question of contributing proportions.
As ATP-CP is intrinsic to the highest intensity muscular contractile dynamics, developing a sprinter’s alactic bioenergetic system, to their genetic ceiling, is of top importance.
No two steps during acceleration occur at the same velocity, as by definition, an acceleration is a change in velocity over time. Acceleration increases up to, and concludes, when maximum velocity is reached, and the point in which maximum velocity finalizes is the point in which the alactic processes cease to be the primary contributing bioenergetic resource.
At this point, anaerobic lactic processes begin to assume greater and greater proportions of the bioenergetic load. The results of which are greater and greater accumulations of blood lactate, along with greater proton production resultant of the muscles’ use of large quantities of ATP [2,7].
In 1961, Peter Mitchell proposed that electron transport and ATP synthesis are coupled by a proton gradient across the inner mitochondrial membrane…[2]. Mitochondrial ATP synthase catalyzes ATP synthesis, utilizing an electrochemical gradient of protons across the inner membrane during oxidative phosphorylation [1].
Every time ATP is broken down to ADP and P(i), a proton is released. When the ATP demand of muscle contraction is met by mitochondrial respiration, there is no proton accumulation in the cell, as protons are used by the mitochondria for oxidative phosphorylation and to maintain the proton gradient in the intermembranous space. It is only when the exercise intensity increases beyond steady state that there is a need for greater reliance on ATP regeneration from glycolysis and the phosphagen system. The ATP that is supplied from these nonmitochondrial sources and is eventually used to fuel muscle contraction increases proton release and causes the acidosis of intense exercise [7].
This proton release that causes the acidosis (not to be confused with lactic acid) is what contributes to the “burning” feeling associated with anaerobic lactic work bouts. However, as elucidated upon by the referenced authors, the historical claim that the accumulation of blood lactate is what causes the “burn” of intense/prolonged exercise is false. To be clear, growing concentrations of blood lactate are closely associated with, yet not the cause of, the high level of muscle discomfort associated with the highest achievable intensities over ~20-60sec durations.
From a proportionality and contribution standpoint, you may objectively state that the shorter the distance over which a sprinter is able to accelerate, the greater the significance of speed endurance training. Alternatively, the longer the distance over which a sprinter is able to accelerate, the greater the significance of maximum velocity training. For this reason, speed endurance training, proportionally, tends to more positively benefit developing female sprinters and younger sprinters in general, as both of these populations, broadly speaking, reach maximum velocity in less time.
Every second of time spent after the sprinter is unable to sustain maximum velocity is a second of time spent in growingly rigorous physiological conditions. Thus, while the development of maximum velocity is the ultimate commodity for a 100m sprinter, any sprinter whose period of post-max velocity sprinting is longer in distance than the distance over which they are able to accelerate is a sprinter who will, in the short term, benefit more greatly from enhancing their ability to sustain the highest percentage of their existing max velocity via speed endurance training.
Alternatively, the more elite a sprinter becomes, the deeper into the race distance they accelerate and, as a consequence, reach a higher maximum velocity. This population of sprinters experiences less physiological stress, as the period over which they battle growing levels of acidosis is shorter in duration than their slower counterparts. Interestingly enough, however, is that the most profound impact on these sprinters’ performance is the continued development of maximum velocity—as incremental as it will be at this point. In these cases, even a relatively small increase in maximum velocity may pose a dramatic reduction in the lactic period.
An IAAF report on the 2009 World Championships in Berlin reveals that Usain Bolt reached his 12.35m/s at 70m and, by comparison, Powell reached his 11.90m/s at 60m [11]. Bolt, therefore, had less than 30m remaining to operate under growing physiological challenges, while Powell had the better part of 40m.
It must be pointed out that the highest maximum velocity does not always amount to the fastest race time. While this may sound peculiar, we must account for the significance of the start and reaction times. Bolt’s 12.35m/s is the highest maximum velocity ever recorded in a 100m competition (some research has him listed at over 12.4m/s at peak velocity). It also accompanied the fastest recorded time in a 100m competition (9.58). Had he stumbled out of the blocks, however, and tripped and fallen to the ground, he still may have recovered and reached 12.35m/s; however, it would have been much closer to the finish. While that would have further reduced the lactic period, it surely wouldn’t have mattered as everyone else would have already long since finished the race.
In regards to what did happen, not only did Bolt register a higher maximum velocity farther into the race, his 60m split bested the 60m world record by a full tenth of a second, and the reduced lactic period allowed him to remain within 2% of his maximum velocity through the tape. The result was the fastest 100m of all time [9].
The “first principles” perspective was utilized to write this article in order to demonstrate one method of preserving objectivity, as well as how to efficiently examine the fundamental basis of any problem solving—which begins with understanding the very structure of the problem itself.
Anyone who is keen to engage in objective discussion of this sort is encouraged to consider a membership in the Conclave on globalsportconcepts.net. This was created to foster unlimited creative freedom in the rational solving of sports training problems and the evolution of coaching as a whole, as inspired by the work of theoretical physicists Neil Turok and David Deutsch.
Definition of Terms
Acceleration: the 2nd derivative of position, 1st derivative of velocity, defined by the change in velocity over the change in time. Defined by meters per second squared (m/s^2).
Angular Acceleration: the rate of change of angular velocity. Described by Radians or Degrees per second squared. (Rad/sec^2 or Deg/sec^2).
Angular Displacement: defined by the angle through which an object moves on a circular path. Described by Radians or Degrees.
Angular Velocity: the rate of change of a rotating object. Described by Radians or Degrees per second (Rad/sec or Deg/sec).
Biodynamics: the study of physical motion or dynamics in living systems.
Bioenergetics: the study of the transformation of energy in living organisms.
Biomotor Outputs: biological motion possibilities regulated by the motor cortex.
Dynamics: the branch of mechanics that deals with the motion of bodies under the action of force (kinematics and kinetics).
First Principles: the fundamental concepts or assumptions on which a theory, system, or method is based.
Force: the product of some mass multiplied by some acceleration. Described by Newtons (kg x m/s^2).
GCT: ground contact time.
Impulse: a change in momentum. Described by Force x Time.
Inertia: the resistance of a physical object to a change in its state of motion. Implicit to Newton’s 1st Law, also known as his law of inertia, which states that objects at rest tend to stay at rest and objects in motion tend to stay in motion (in the same direction and at the same speed) unless acted upon by an unbalanced force.
Jerk: the 3rd derivative of position, 2nd derivative of velocity, 1st derivative of acceleration, defined by the change in acceleration over the change in time. Described by meters per second cubed (m/s^3).
Kinematics: the study of motion, change in position (and its derivatives: velocity, acceleration, and jerk), without consideration of mobilizing forces.
Kinetics: the study of motion and its mobilizing forces.
Lever: A simple machine consisting of a rigid bar pivoted on a fixed point and used to transmit force.
Lever Arm: the perpendicular distance between the axis of rotation and the line of action of the force.
Momentum: a quantity of a moving object’s motion, described by mass x velocity.
Quantitative Units of Measurement: length (meter), mass (kilogram), time (second).
Sprint Training: physical training intended to improve an athlete’s ability to sprint faster over a given distance.
Vector: in physics, any quantity that possess both a magnitude and direction.
Velocity: the first derivative of position characterized by change in position over change in time. Described by meters per second (m/s).
Since you’re here…
…we have a small favor to ask. More people are reading SimpliFaster than ever, and each week we bring you compelling content from coaches, sport scientists, and physiotherapists who are devoted to building better athletes. Please take a moment to share the articles on social media, engage the authors with questions and comments below, and link to articles when appropriate if you have a blog or participate on forums of related topics. — SF
[mashshare]
References:
- ATP5B ATP synthase, H+ transporting, mitochondrial F1 complex, beta polypeptide [Homo sapiens (human)] Gene ID: 506, updated on 19-Mar-2017.
- Berg J.M., Tymoczko J.L., Stryer L. Biochemistry. 5th edition. New York: W H Freeman; 2002. Section 18.4, “A Proton Gradient Powers the Synthesis of ATP.”
- Čoh M., Tomažin K., Štuhec S. “The Biomechanical Model of the Sprint Start and Block Acceleration.” University of Ljubljana, Faculty of Sport, Ljubljana, Slovenia. FACTA UNIVERSITATIS Series: Physical Education and Sport Vol. 4, No 2, 2006, pp. 103 – 114
- Mendiguchia J., Martinez-Ruiz E., Edouard P., Morin J.B., Martinez-Martinez F., Idoate F., Mendez-Villanueva A. “A Multifactorial, Criteria-based Progressive Algorithm for Hamstring Injury Treatment.” Med Sci Sports Exerc. 2017 Mar 8. PMID 28277402
- Morin J.B., Edouard P., Samozino P. “New Insights into Sprint Biomechanics and Determinants of Elite 100m Performance.” New Studies in Athletics. 2013.
- Morin J.B., 2017 IOC workshop: “Sprint Acceleration Force-Velocity Profile and Hamstring Injury Management: Win-Win?” YouTube.com. Mar 2017.
- Robergs R. A., Ghiasvand F., Parker D. “Biochemistry of exercise-induced metabolic acidosis.” American Journal of Physiology. 2004.
- Smith, J. “Applied Physiology- Anaerobic Supply Mechanisms.” Freelapusa. 2014.
- Smith, J. Applied Sprint Training. 2014.
- Smith, J. The Governing Dynamics of Coaching. 2016.
- Hommel, H. “Biomechanical Analysis of Selected Events at the 12th IAAF World Championships in Athletics.” Berlin, Aug. 2009.